The Role of CRISPR-Cas9 in Treating Genetic Diseases
- dorottya.szasz
- Aug 12, 2024
- 10 min read
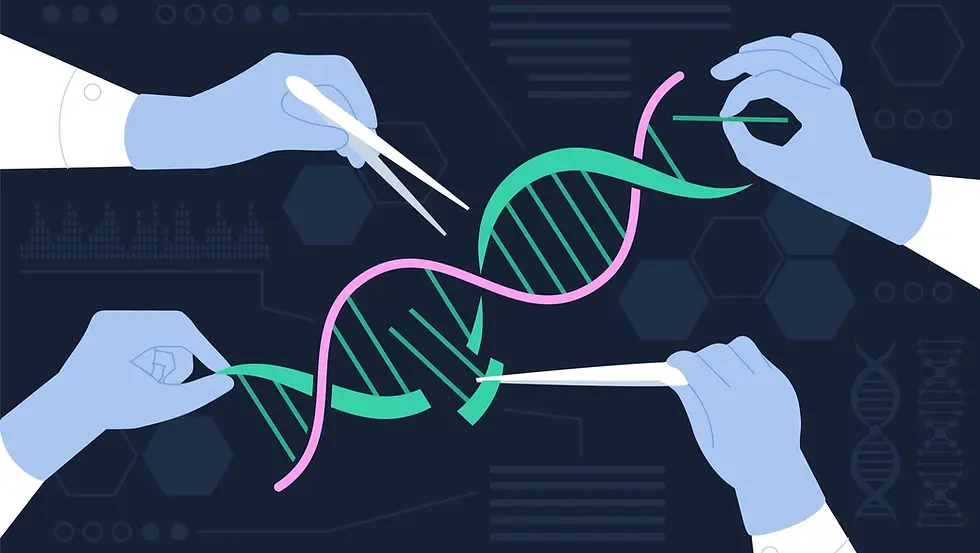
1. Introduction to Genetic Diseases and complex interactions
Monogenic disorders, such as cystic fibrosis and sickle cell anemia, result from a mutation in a single gene. On the other hand, polygenic disorders like diabetes and heart disease are influenced by the combined effects of multiple genes, as well as environmental and lifestyle factors. The significance of genetic diseases extends beyond their impact on individual health. They play a crucial role in the field of genetics research, as they provide valuable insights into gene function and inheritance patterns. By studying the genetic basis of these disorders, scientists can develop better diagnostic tools, treatments, and preventive measures. In addition to their biological implications, genetic diseases also pose social and ethical considerations. The availability of genetic testing has opened up opportunities for individuals and families to make informed decisions about their health, reproduction, and family planning. However, it also raises concerns such as privacy, discrimination, and the use of such information in societal contexts. As our understanding of genetics continues to advance, so does our ability to detect and treat genetic diseases. Genetic counseling, precision medicine, and gene therapy are among the innovative approaches that hold promise in the field of genetic disease management. Furthermore, ongoing research aims to uncover new therapies and interventions that can effectively address the underlying causes of these conditions. By raising awareness, fostering collaborations, and investing in genetic research, we can continue to make significant progress in the understanding and management of genetic diseases. Through a multidisciplinary approach that combines genetics, genomics, medicine, and public health initiatives, we can strive towards the prevention, early diagnosis, and improved treatment of these conditions. Ultimately, our collective efforts have the potential to transform the lives of individuals and families affected by genetic diseases, leading to a healthier and more inclusive future for all.(Cai et al., 2016). Monogenic disorders are rare and one-third of these diseases are of autosomal recessive type. The most common autosomal recessive genetic disease is cystic fibrosis (CF), an ion channel disorder caused by mutations in the cystic fibrosis transmembrane conductance regulator gene (CFTR). A common deletion of phenylalanine at codon 508 (F508del) accounts for ~70% mutations (Khan et al., 2018). CF affects epithelial tissues in the lungs, intestine and pancreas and is characterized by abnormal ion transport, thickened mucus, persistent lung infections, and eventually respiratory failure.
Duchenne muscular dystrophy (DMD) is an X-linked form of muscular dystrophy, caused by mutations in the dystrophin gene (DMD). Mutations in this 2.4Mb gene lead to the absence of dystrophin protein, compromised stability of muscle cell membranes, myofiber degeneration, and eventually loss of skeletal and cardiac muscles. DMD primarily affects males and occurs at a frequency of 1 in 3500 newborn boys, with a life expectancy of 20s-30s. Spinal muscular atrophy (SMA) is a common recessive disease, characterized by progressive and selective degeneration of motor neurons in the anterior horn of the spinal cord. This leads to weakness and atrophy of proximal muscles, impaired ambulation, and respiratory weakness. SMA is caused by homozygous deletions or mutations of the SMN1 gene that are accompanied by the presence of SMN2 gene. SMA has several phenotypes and two severe ones (Type 0 and Type I) occur in babies who do not reach the milestone of sitting. SMA is the leading genetic cause of infant mortality, occurring in approximately 1 in 6000-10000 births.
1.1. Definition and Types of Genetic Diseases
Genetic diseases, also referred to as inherited diseases, are diseases that are caused by abnormalities in an individual's genetic material. An abnormality can be a mutation in the DNA sequence, a missing or extra chromosome, or rearrangements of chromosomes such as translocations. Commonly, genetic diseases may be classified based on inheritance modes into chromosomal abnormalities (or chromosomal disorders), monogenic heritable diseases (or Mendelian diseases), and complex genetic diseases (or multifactorial disorders). Chromosomal abnormalities involve alterations of a whole chromosome or segments within a chromosome, which can lead to an alteration of the chromosome number, such as Down syndrome (Cai et al., 2016). Monogenic heritable diseases are caused by a mutation in a single gene. These diseases may be transmitted as dominant or recessive. In a dominantly inherited disease, only one copy of the gene has to carry a mutation (which may be a deletion), for a person to exhibit the disease. In a recessively inherited disease, a person has to possess mutations in both copies of the gene. Most of the monogenic heritable diseases are recessively inherited diseases, such as cystic fibrosis (CF), sickle cell anemia (SCA), and Tay Sachs disease (TSD) (Khan et al., 2018).
Complex genetic diseases result from interaction between multiple genes and environmental factors, which is the most common disease class in the general population. Most psychiatric/neurological diseases belong to this category. Well-known examples include schizophrenia (SCZ), autism (AUTS), and epilepsy (EPI) families. Other diseases include Alzheimer and cardiovascular diseases. Diseased individuals often exhibit variations in multiple risk genes and archaic variants with subtle effects in each single gene.
2. Introduction to CRISPR-Cas9
Clustered regularly interspaced short palindromic repeats (CRISPR)-CRISPR-associated protein (Cas) systems are adaptive immune systems used by many bacteria and archaea to fight off foreign DNA, most of which is derived from viruses. These systems have also been identified as a group of small non-coding RNAs with homologous sequences and the accompanying Cas proteins. This diversity of sequences and associated proteins led to the classification of CRISPR-Cas into two broad classes, six types, and numerous subtypes. Of particular interest is the type II CRISPR-Cas system which has been engineered for use as a programmable nuclease technology in mammalian cells. Although plasmids have been commercialized for use in mammalian cell lines and primary neurons, there is still a need for better genomic target design vectors that can be utilized across multiple cell lines. DNA-free approaches targeted to specific genomic loci have recently been developed, and CRISPR-Cas bacterial species with different Cas proteins and tracrRNAs may provide insight into the evolution and advancement of the CRISPR-Cas technology (E Humphrey & L Kasinski, 2015).
A CRISPR-Cas system consists of two main components: the Cas genes, and the CRISPR cluster with its repeat-spacer sequences. The CRISPR array exhibits a unique arrangement of short repeat sequences 239-371 bp in length and long interspersed unique or spacer sequences, which are homologous to the foreign DNA. The foreign viral sequences captured by the CRISPR-Cas system17 are inserted between CRISPR regions in the genome of the host organism. Upon reinfection, one or more CRISPR-associated (Cas) proteins become activated, and the foreign CRISPR array is transcribed as a long pre-crRNA. The pre-crRNA then undergoes processing by Cas proteins and/or RNase III to generate individual CRISPR RNAs (crRNAs). The crRNAs are then bound by transactivating CRISPR RNAs (tracrRNAs), ensuring the processing of the precursor CRISPR RNA and recruitment of an endonuclease at the 5’ end. Each crRNA:tracrRNA duplex directs an endonuclease to its specific target DNA in a sequence-dependent manner. The effector complexes elucidated for class I systems comprise a multi-protein complex with crRNA as a common component. On the other hand, type II CRISPR-Cas systems utilize a single Cas9 protein that is both functionally and physically coupled with crRNA/tracrRNA (Janik et al., 2020).
2.1. Basic Mechanism
In the bacteria Streptococcus pyogenes, a collection of DNA repeats were discovered, found with sequence homology with exogenous DNA of bacteriophages or plasmids. It was later demonstrated that the genetic elements that created this adaptive defense mechanism were Cas (CRISPR-associated) proteins and RNAs called crRNAs (CRISPR RNAs) (Liu et al., 2017). The classical CRISPR/Cas systems are categorized into two major classes, class 1 containing types I, III, and IV, and class 2 containing types II, V, and VI. Most of the research interest focuses on the class 2 type II CRISPR/Cas9 (Whinn et al., 2019). This system requires the Cas9 protein, a 3-part RNA transcript, the crRNA (CRISPR RNA) complementary to the target strand, the tracrRNA (trans-acting crRNA) hybridizing with crRNA, and the RNase III cutting the long RNA transcript to two smaller RNAs in a 1:1 ratio. Upon binding, a triplex is formed where the helicase activity of Cas9 unwinds the target DNA and the crRNA targets it via complementary base pair hybridization. Further, the presence of PAM (protospacer adjacent motif) is needed, specific 2-6 nucleotide sequences on the target DNA recognized by Cas9. Initially, the Cas9 associated with non-target DNA is tested for the PAM presence, and only those strands possessing the PAMs are targeted for subsequent matches to the guide RNA. The limited activity of Cas9 on the non-target DNA is crucial as it limits the off-target cleavage of the genome. Thus, the type II Cas9 systems have all the elements for efficient genome editing.
3. Applications of CRISPR-Cas9 in Genetic Disease Treatment
(Cai et al., 2016)
Since Cas9 was equated to the customizable endonucleases, technological advances for safe and effective delivery of CRISPR-Cas9 systems have made exponential progress. According to the recent clinical trials registered in the ClinicalTrials.gov database, over a dozen CRIPR-Cas9 based clinical trials have been launched, primarily targeting hematopoietic cells, thus exploring diverse dosing protocols encompassing co-administration and successive delivery approaches. Additionally, solid tumors born of liver cancer and other solid cancer will be explored (Khoshandam et al., 2023). In the hope of ameliorating genetic diseases, the first CRISPR-Cas9 based clinical trial has been conducted to disrupt the gene encoding the Cu/Zn superoxide dismutase enzyme to treat familial amyotrophic lateral sclerosis. Furthermore, safety issues were raised due to possible off-target events with concurrent editing of other undesirable loci, including tumor suppressors.
3.1. Gene Editing in Inherited Disorders
Genome editing holds great promise for correcting disease-causing mutations in all types of inherited disorders. Gene therapy offers the tantalizing prospect of a cure for otherwise fatal diseases, and recent trials in pro-funding severe combing immunodeficiency (SCID) diseases have shown safety with several successful cases. However, the ability of today’s gene therapies to address ‘invisible’ diseases due to single-base changes or unexpressed mutations has been limited. Genome editing, and particularly the CRISPR-Cas9 system, has the potential to overcome current gene replacement limitations. This local approach to the easily manipulated target sequence offers a powerful means to cure genetic diseases in a variety of tissues and ages by stabilizing the genetic code (Gaskell1 & Abualhaj2, 2018).
CRISPR-Cas9 is a powerful tool for gene editing due to its ease of design, stability, and efficiency. While the biochemistry of CRISPR-Cas9 is reasonably well-understood, unintended consequences (off-target editing) can lead to unwanted effects. There are two approaches to limit off-target effects: optimizing the Cas9 protein and developing a robust delivery system. Although Cas9 variants with reduced off-target activity have been developed, the majority of ongoing developments focus on the co-delivery of a CAS9 variant with a sgRNA library targeting all the predicted off-targets of the specific sgRNA used in the respective experiment (Khoshandam et al., 2023).
3.2. Potential Therapies for Common Genetic Diseases
The potential of CRISPR/Cas9 for treating some of the most common genetic diseases, which for the most part are single nucleic acid changes, will be discussed. The diseases treated include attempts at creating models for diseases in human embryonic stem cells or at using CRISPR/Cas9 in animal models, which include Duchenne muscular dystrophy, Huntington’s disease, cystic fibrosis, sickle cell anemia, β-thalassemia, hemophilia, Usher syndrome, alpha-1 antitrypsin (a1AT) deficiency, and fragile X syndrome (Cai et al., 2016) ; (Khan et al., 2018). As the diseases considered have somewhat different inheritance patterns and consequences for the affected individuals, a closer examination of each is warranted.
CRISPR/Cas9 has been the subject of an enormous increase in research activity since its initial description as a microbial adaptive immune system in 2012. Over the past 5 years, it has emerged as an extremely valuable and popular tool for genome engineering and gene regulation in microorganisms, plants, and animals. More recently, attention began to be directed towards its potential use for treating human disease. Because of the potential for curing diseases, this area has been intensely pursued and resultant studies on a variety of diseases are emerging rapidly.
4. Challenges and Limitations
Advances in clustered regularly interspaced short palindromic repeats (CRISPR)-associated systems (Cas) technologies have greatly enhanced genetic editing applications as well as the examination of biological functions of any genes, genotyping of pre-inserted transgenes, and creation of engineered laboratory animals for the generation of humanized animal models for biomedical research. Moreover, these technologies have revolutionized the gene editing field, making a huge transition for biotechnology applications especially in specimen or pathogen screening and inspection, genomic interrogation and genome reconstruction, potential genetic breeding technologies in aquaculture, plants, livestock, and production of gene-edited agricultural commodities and food (Liu et al., 2021).
Recently, these CRISPR-Cas gene editing technologies have been widely considered as a novel class of therapeutic technologies for disease treatment. Many inherited genetic disorders stemming from single nucleotide mutations or small fragments of genetic variants in protein coding regions exist and more than 400 have been considered mainly monogenic Mendelian diseases, causing a tremendous burden on the affected individuals, family, and society. Hence, it is urgent and important to discover new effective methodologies for treating these single-gene diseases. Compared with emerging next-generation gene-editing tools (e.g., base or prime editors), CRISPR-Cas systems possess major advantages in gene editing, including high accuracy, efficiency, economy, flexibility, and versatility, and have been recognized as a feasible approach for treating single-gene diseases. Such CRISPR-Cas therapeutic treatments aim to directly revert the underlying disease-causing mutations by restoring the normal gene functions, thereby curing the genetic diseases. In addition to the enormous efforts focusing on single-gene diseases, it is also feasible to treat some recessive genetic diseases by supplying therapeutically effective wild-type mRNAs or normal alleles of the mutated genes for restoring gene functions through CRISPR-Cas-mediated technology.
5. Future Directions and Potential Developments
Although much of CRISPR-Cas9 research targets genetic disease, there is growing interest in using the technology to combat other diseases. CRISPR-Cas9-based strategies have been increasingly applied to human diseases including cancer, genetic disorders, infectious diseases, respiratory virus infections, inflammatory diseases, and neurological disorders. For example, CRISPR-Cas9 was used to knockout the gene CTLA-4 to engineer an anti-PD-1 chimeric antigen receptor T cell (CAR-T) siRNA to treat cancer (Liu et al., 2021). In a different study, CRISPR-Cas9 was used along with other technologies to decrease the viral load associated with the severe acute respiratory syndrome coronavirus 2 (SARS-CoV-2) pathogen (Khan et al., 2018). These CRISPR-Cas9-based approaches are not just therapy-oriented but can also be used for disease modeling, leading to an improved understanding of various infectious and genetic diseases.
References:
Cai, L., L. Fisher, A., Huang, H., & Xie, Z. (2016). CRISPR-mediated genome editing and human diseases. ncbi.nlm.nih.gov
Khan, S., Shahid Mahmood, M., ur Rahman, S., Zafar, H., Habibullah, S., khan, Z., & Ahmad, A. (2018). CRISPR/Cas9: the Jedi against the dark empire of diseases. ncbi.nlm.nih.gov
E Humphrey, S. & L Kasinski, A. (2015). RNA-guided CRISPR-Cas technologies for genome-scale investigation of disease processes. ncbi.nlm.nih.gov
Janik, E., Niemcewicz, M., Ceremuga, M., Krzowski, L., Saluk-Bijak, J., & Bijak, M. (2020). Various Aspects of a Gene Editing System—CRISPR–Cas9. ncbi.nlm.nih.gov
Liu, X., Wu, S., Xu, J., Sui, C., & Wei, J. (2017). Application of CRISPR/Cas9 in plant biology. ncbi.nlm.nih.gov
Whinn, K., M van Oijen, A., & Ghodke, H. (2019). Spy-ing on Cas9: Single-molecule tools reveal the enzymology of Cas9. [PDF]
Khoshandam, M., Soltaninejad, H., Mousazadeh, M., Ali Hamidieh, A., & Hosseinkhani, S. (2023). Clinical applications of the CRISPR/Cas9 genome-editing system: Delivery options and challenges in precision medicine. ncbi.nlm.nih.gov
Gaskell1, D. & Abualhaj2, M. (2018). Advances in Gene Therapy for Human Genetic Diseases Based on Crispr/Cas9 Gene Editing Technology. [PDF]
Liu, W., Li, L., Jiang, J., Wu, M., & Lin, P. (2021). Applications and challenges of CRISPR-Cas gene-editing to disease treatment in clinics. ncbi.nlm.nih.gov
Kommentare